M. R. S. and S. P. L. contributed equally to this study.
Sleep spindles and mobile phones
Sleep EEG alterations: effects of different pulse-modulated radio frequency electromagnetic fields
Article first published online: 12 APR 2011
DOI: 10.1111/j.1365-2869.2011.00918.x
© 2011 European Sleep Research Society
Additional Information(Show All)
How to CiteAuthor InformationPublication History
How to Cite
SCHMID, M. R., LOUGHRAN, S. P., REGEL, S. J., MURBACH, M., BRATIC GRUNAUER, A., RUSTERHOLZ, T., BERSAGLIERE, A., KUSTER, N. and ACHERMANN, P. (2012), Sleep EEG alterations: effects of different pulse-modulated radio frequency electromagnetic fields. Journal of Sleep Research, 21: 50–58. doi: 10.1111/j.1365-2869.2011.00918.x
Publication History
- Issue published online: 20 JAN 2012
- Article first published online: 12 APR 2011
- Accepted in revised form 12 March 2011; received 1 November 2010
- Abstract
- Article
- References
- Cited By
Keywords:
- cognition;
- electroencephalogram;
- mobile phone;
- spectral power;
- spindle frequency
Summary
- Top of page
- Summary
- Introduction
- Materials and Methods
- Results
- Discussion
- Acknowledgements
- Conflicts of Interest
- References
Previous studies have observed increases in electroencephalographic power during sleep in the spindle frequency range (approximately 11–15 Hz) after exposure to mobile phone-like radio frequency electromagnetic fields (RF EMF). Results also suggest that pulse modulation of the signal is crucial to induce these effects. Nevertheless, it remains unclear which specific elements of the field are responsible for the observed changes. We investigated whether pulse-modulation frequency components in the range of sleep spindles may be involved in mediating these effects. Thirty young healthy men were exposed, at weekly intervals, to three different conditions for 30 min directly prior to an 8-h sleep period. Exposure consisted of a 900-MHz RF EMF, pulse modulated at 14 Hz or 217 Hz, and a sham control condition. Both active conditions had a peak spatial specific absorption rate of 2 W kg−1. During exposure subjects performed three different cognitive tasks (measuring attention, reaction speed and working memory), which were presented in a fixed order. Electroencephalographic power in the spindle frequency range was increased during non-rapid eye movement sleep (2nd episode) following the 14-Hz pulse-modulated condition. A similar but non-significant increase was also observed following the 217-Hz pulse-modulated condition. Importantly, this exposure-induced effect showed considerable individual variability. Regarding cognitive performance, no clear exposure-related effects were seen. Consistent with previous findings, our results provide further evidence that pulse-modulated RF EMF alter brain physiology, although the time-course of the effect remains variable across studies. Additionally, we demonstrated that modulation frequency components within a physiological range may be sufficient to induce these effects.
Introduction
- Top of page
- Summary
- Introduction
- Materials and Methods
- Results
- Discussion
- Acknowledgements
- Conflicts of Interest
- References
Mobile phones have become important devices of modern communication. As a result of the widespread increase in use of this technology, concerns have been raised regarding the potential impact on human health, particularly on the CNS. Despite numerous studies having been performed to address this issue, it still remains unclear how and to what extent the human brain is affected by radio frequency electromagnetic fields (RF EMF) emitted by mobile phones.
Several experiments have revealed short-term changes in electroencephalographic (EEG) spectral power during both waking (Croft et al., 2002, 2008, 2010; Curcio et al., 2005; Regel et al., 2007a) and sleep (Borbély et al., 1999; Huber et al., 2000, 2002; Loughran et al., 2005; Regel et al., 2007b) in conjunction with exposures to mobile phone-like RF EMF. Particularly in relation to sleep, which is also the focus of the current study, it should be noted that some studies have failed to find any RF EMF exposure-related effects on the EEG (Fritzer et al., 2007; Wagner et al., 1998, 2000). However, when study and statistical methodologies are taken into account, as well as dosimetric and exposure parameters, the studies that have given greater emphasis to these important factors have consistently shown RF EMF-induced effects on EEG spectral power during sleep (Borbély et al., 1999; Huber et al., 2000, 2002; Loughran et al., 2005; Regel et al., 2007b). This increase in EEG power has been shown during and following RF EMF exposures, and has mostly been observed in the spindle frequency range during non-rapid eye movement (NREM) sleep (approximately 11–15 Hz). One recent study also suggested that the increase in EEG spectral power may be dose dependent (Regel et al., 2007b). Other results indicate that pulse modulation of the RF EMF, as employed by the most widely used system for mobile telephony (Global System for Mobile Communications, GSM), is crucial to induce changes in brain activity, as continuous wave exposure at the same intensities did not induce any effects on the EEG (Huber et al., 2002; Regel et al., 2007a). Despite these consistent exposure-related enhancements of EEG power in the spindle frequency range during sleep, it should be noted that the time-course of the effect has been somewhat variable across studies, with effects observed at several different time-points, as well as throughout the sleep period (Borbély et al., 1999; Huber et al., 2000, 2002; Loughran et al., 2005; Regel et al., 2007b). Overall, these previous studies indicate that pulse-modulated RF EMF exposure does have an influence on the sleep EEG, which is in line with two recent reviews that also conclude there is sufficient evidence of exposure-related effects (Valentini et al., 2007; Van Rongen et al., 2009).
In addition to changes in brain activity, changes in cognitive performance in response to RF EMF exposures have previously been reported, most notably in relation to reaction speed and accuracy of performance in various cognitive tasks. However, the findings have been largely inconsistent, with some studies showing improvements (e.g. Koivisto et al., 2000; Preece et al., 1999), some showing impairments (e.g. Regel et al., 2007b), and others finding no measureable effects (e.g. Haarala et al., 2003, 2004). These conflicting reports have led to uncertainty regarding potential effects on cognition; however, a recent meta-analysis by Valentini et al. (2010) concluded that mobile phone-like RF EMF exposure does not seem to cause cognitive effects.
Overall, while no consistent effects on cognition have been observed, studies have repeatedly shown similar effects of mobile phone-like RF EMF exposures on the EEG. The observation that pulse-modulated, but not continuous wave, RF EMF affects the EEG in sleep points towards a non-thermal biological mechanism in which pulse modulation plays an important role. However, it remains unclear which components of the RF EMF could be responsible for the changes seen in EEG spectral power, or whether the strength of certain low-frequency modulation components or the crest factor (the ratio between pulse peak power and time-averaged power) of the applied field are key elements to induce an effect.
The present study aimed to investigate which field parameters may be responsible for the previously reported results on electrical brain activity during sleep, while also providing an indication of the underlying mechanisms involved. We hypothesized that pulse-modulation components in the sleep spindle frequency range (e.g. 14 Hz) could act as potential mediators, as the majority of previous studies found an influence of RF EMF exposure in this frequency range. Additionally, we investigated pulse modulation at 217 Hz, as this is the strongest component of the GSM signal and has been consistently included in the exposure signal of previous studies reporting effects on EEG spectral power. Cognitive tasks were also implemented during exposure with the aim to address previous inconclusive results regarding the effects of exposure to pulse-modulated RF EMF on cognition.
Materials and Methods
- Top of page
- Summary
- Introduction
- Materials and Methods
- Results
- Discussion
- Acknowledgements
- Conflicts of Interest
- References
Subjects
Thirty young healthy men, aged between 20 and 26 years [mean age 23.0 ± 0.3 years (±SEM)] were recruited by advertisement at the University of Zurich and ETH Zurich. All subjects were right-handed, non-smokers and free of sleep complaints, drugs or medication. All participants owned a mobile phone and reported to use it <2 h per week (average use 41.1 ± 6.2 min per week).
A screening night was performed to identify and exclude subjects with sleep disturbances (sleep apnoea and nocturnal myoclonus) and sleep efficiency of <80%. Participants had to abstain from caffeine and alcohol consumption, and adhere to regular bedtimes (8 h, according to their scheduled bedtime in the sleep lab) starting 3 days before the study. Compliance was controlled by breath alcohol tests, wrist-worn actimeters and sleep logs. On all study days physical exercise had to be avoided, and on the exposure days mobile phone calls were prohibited. The study protocol was approved by the cantonal ethical committee, and participants gave their written informed consent and were recompensed upon completion of the study.
Study procedure
The study was carried out in the sleep laboratory of the Institute of Pharmacology and Toxicology, University of Zurich. The protocol consisted of six study nights (three exposure nights at weekly intervals, each preceded by an adaptation night). Because only two persons could be exposed simultaneously, participants were divided into pairs and scheduled at different times for the exposure. Therefore, respective bedtimes were either from 22:40 to 06:40 hours or 23:20 to 07:20 hours, with each participant assigned to the same time schedule for exposure and sleep throughout the study. During exposure subjects performed three different cognitive tasks while their heads were positioned between two planar antennas. Exposure lasted 30 min and ended 10 min before subjects had to go to bed. After exposure subjects were asked to indicate whether they were able to perceive a field. Prior to bedtime and after waking up in the morning, subjects filled in questionnaires rating their current mood state and wellbeing on a visual analogue scale.
Exposure conditions
Three exposure conditions were applied in a partially balanced, randomized double-blind crossover design: (i) a pulse-modulated RF EMF at 14 Hz (crest factor = 30.95, pulse width = 2.3 ms); (ii) a pulse-modulated RF EMF at 217 Hz (crest factor = 8, pulse width = 0.577 ms); and (iii) a sham condition. A broader pulse width was used in the 14-Hz condition due to safety considerations. Both active field conditions were applied at the left side of the subject’s head with a carrier frequency of 900 MHz and a time-average peak spatial specific absorption rate (SAR, 10 g average) of 2 W kg−1 (for detailed SAR distribution, see Huber et al., 2003, 2005; Boutry et al., 2008; note that the illustrated exposed hemisphere is the opposite to that of the current study, and that 0 dB now equals 2 W kg−1), corresponding to the exposure limit for the general public (International Commission on Non-Ionizing Radiation Protection., 1998). Higher harmonics were present due to the rectangular pulse structures (for details on signal characteristics, see Fig. 1).

Figure 1. Signal characteristics of the two applied field conditions and their corresponding amplitude spectra. The 10 g peak spatial specific absorption rate (SAR) was 2 W kg−1 for both signals. (a) Pulse structures of the 14-Hz pulse-modulated RF EMF. Pulse width was 2.3 ms with a SAR peak of 61.9 W kg−1. The crest factor (ratio of pulse peak power to average power) was 30.95. (b) Amplitude spectra of the envelope (normalized to 100 at 0 Hz, as in Huber et al., 2005) of the 14-Hz pulse-modulated (pm) RF EMF. (c) Pulse structures of the 217-Hz pulse-modulated RF EMF. Pulse width was 0.577 ms with a SAR peak of 16 W kg−1 and crest factor of 8. (d) Amplitude spectra of the envelope of the 217-Hz pm RF EMF.
To ensure a well-defined position with respect to the two planar antennas, the subjects’ heads were positioned between two plates such that the centre of the antenna was 42 mm vertically above the ear canal at a distance of 115 mm from the head (Huber et al., 2000, 2003). Electrode leads were placed horizontally to the emitted field in order to minimize any possible interference of the RF EMF. To avoid acoustic perception of the active field conditions, a brown noise (spectral density proportional to 1/f2) was generated by a loudspeaker in the exposure room. Average sound pressure levels were estimated to be <35 dB without noise (below threshold of the device), and 43 ± 2 dB with applied brown noise at the level of the subjects’ heads.
Polysomnographic recordings
During 8 h of nighttime sleep we continuously recorded the EEG (derivation C3A2), electrooculogram, electromyogram (EMG; mental or submental) and electrocardiogram (ECG) with a polygraphic amplifier Artisan (Micromed, Mogliano Veneto, Italy). The analogue signals were conditioned by a high-pass filter (EEG: −3 dB at 0.15 Hz; EMG: 10 Hz; ECG: 1 Hz) and an anti-aliasing low-pass filter (−3 dB at 67.2 Hz), digitized at 256 Hz, and transmitted via fibre-optic cables to a computer, running the software Rembrandt DataLab (Version 8.0; Embla Systems, Broomfield, CO, USA).
Visual scoring of 20-s epochs was performed according to the criteria of Rechtschaffen and Kales (1968). Sleep cycles were defined according to Feinberg and Floyd (1979). EEG power spectra of consecutive 20-s epochs (FFT routine, Hanning window, averages of five 4-s epochs) were computed, and artefacts were removed both visually and with a semi-automatic procedure (Huber et al., 2000). The frequency resolution was 0.25 Hz, and frequencies between 0.75 and 20 Hz were analysed.
Mean power spectra of NREM and stage 2 sleep (for the first four NREM sleep episodes) were calculated and analysed with linear mixed models (SAS 9.1.3; SAS Institute, Cary, NC, USA), presuming an identical intraclass correlation for all subjects. We included the factors Condition (sham, 14-Hz and 217-Hz pulse modulation) and Order (sequence of applied conditions), as well as their interaction. Where the factor Condition or the interaction Condition × Order was significant, post hoc paired t-tests were performed (level of significance α < 0.05). Additionally, analyses of the temporal evolution of the spectral power in the two specific frequency bands where an effect was observed (11–11.5 Hz, 12.75–13 Hz) were performed across the first four NREM sleep episodes. The linear mixed model included the factors Condition, Episode (1–4) and the Condition × Episode interaction.
As spindle peak locations in the power density spectra vary considerably among individuals, individual peaks were detected in the range from 10 to 15 Hz based on whole-night power density spectra of stage 2 sleep. If more than one peak was present (observed in five of 29 participants), the peak with the higher frequency was selected (due to effects most commonly being observed in the fast spindle frequency range). Corresponding spindle peak power was calculated in stage 2 sleep of NREM sleep episodes as power in the range of ±0.5 Hz around the peak for each subject.
For rapid eye movement (REM) sleep, average power spectra were calculated and analysed for each episode with linear mixed models using the factors Condition, Order and the corresponding interaction. Subjects who had <5 min of artefact-free epochs within a REM sleep episode were excluded for that episode. Furthermore, outliers with relative EEG power changes of more than 100% compared with sham were excluded (based on the most consistent changes in NREM sleep in the current study being of a magnitude of 80% or less). The first REM sleep episode was not considered, due to an insufficient number of subjects reaching the 5-min criterion (n = 9). Twenty subjects contributed to episodes 2 and 4, and 21 to episode 3.
Sleep variables as derived from visual scoring (Tables 1 and 2) were analysed with a linear mixed model anova including the factors Condition, Order and their interaction. One subject was excluded from the sleep analysis due to insufficient sleep efficiency in all three exposure nights. Heart rate was derived from R–R intervals detected in the ECG and averaged for each NREM and REM sleep episode. Analysis was performed as for the power spectral data.
Sham | 14-Hz pm | 217-Hz pm | |
---|---|---|---|
| |||
Total sleep time (min) | 456.5 (2.4) | 454.3 (2.1) | 456.3 (2.5) |
Sleep latency (min) | 13.8 (2.2) | 14.4 (1.7) | 15.2 (2.7) |
REM sleep latency (min) | 73.0 (2.8) | 71.2 (2.7) | 68.7 (2.3) |
Waking after sleep onset (min) | 3.5 (1.5) | 4.7 (1.5) | 2.2 (0.6) |
Stage 2 (min) | 200.9 (5.9) | 201.0 (4.9) | 206.9 (5.6) |
Slow wave sleep (min) | 120.3 (5.5) | 119.8 (4.8) | 118.6 (3.8) |
REM sleep (min) | 121.6 (4.1) | 120.1 (2.9) | 116.8 (4.9) |
Movement time (min) | 6.9 (0.6) | 6.5 (0.6) | 6.5 (0.5) |
Sleep efficiency (%) | 95.0 (0.5) | 94.6 (0.4) | 95.0 (0.5) |
Sham | 14-Hz pm | 217-Hz pm | |
---|---|---|---|
| |||
Cycle 1 | |||
NREMS (min) | 73.0 (2.8) | 71.2 (2.7) | 68.7 (2.3) |
REMS (min) | 15.7 (2.7) | 13.8 (2.2) | 14.8 (2.0) |
Stage 2 (%) | 24.2 (1.7) | 23.7 (1.6) | 23.6 (1.9) |
Cycle 2 | |||
NREMS (min) | 76.5 (2.9) | 76.8 (2.5) | 79.5 (2.3) |
REMS (min) | 30.8 (2.5) | 30.8 (2.4) | 29.7 (2.9) |
Stage 2 (%) | 52.8 (4.1) | 50.4 (3.6) | 53.5 (3.1) |
Cycle 3 | |||
NREMS (min) | 75.4 (2.6) | 75.2 (2.6) | 71.5 (2.3) |
REMS (min) | 34.3 (2.7) | 34.1 (3.1) | 35.3 (3.1) |
Stage 2 (%) | 76.0 (2.9) | 72.9 (2.6) | 74.7 (2.7) |
Cycle 4 | |||
NREMS (min) | 61.0 (1.8) | 61.1 (1.9) | 61.8 (2.5) |
REMS (min) | 39.8 (3.5) | 44.9 (3.4) | 40.7 (4.8) |
Stage 2 (%) | 74.9 (3.3) | 80.4 (3.6) | 76.6 (3.1) |
Cognitive tasks
During the 30 min of exposure, subjects performed three different cognitive tasks [‘simple reaction time task’ (SRT); ‘2-choice reaction time task’ (CRT); ‘N-back task’ (N-back)]. To assess possible changes that might occur during exposure, the 30 min was divided into two 15-min sessions in which each task was presented twice in a fixed order (SRT, CRT, 1-, 2-, 3-back). In the SRT, subjects had to press a ‘0’ on the response box with the right index finger whenever a ‘0’ appeared on the screen. During the CRT, they had to press a ‘J’ or ‘N’ button (right index and middle finger) on the response box whenever ‘JA’ (yes) or ‘NEIN’ (no) was shown on the screen. The N-back task included three levels showing a one-by-one random sequence of consonants, and subjects had to compare the current letter with the letter one, two or three trials back and respond by pressing ‘J’ for same letters or ‘N’ for different letters. The cognitive tasks were applied and analysed as described by Regel et al. (2007b). As residuals of speed [1/reaction time, (1/s)] for SRT were not normally distributed, a non-parametric Wilcoxon-signed-rank test was performed to compare the two exposure conditions with sham for sessions 1 and 2, and the difference between the two sessions. For the CRT and N-back a linear mixed model anova was performed, which included the factors Condition (sham, 14 Hz, 217 Hz), Session (1, 2), Order (sequence of applied conditions), as well as interaction effects. Significant differences were further analysed by post hoc paired t-tests. Accuracy (percentage of correct answers) for the CRT and N-back tasks was statistically analysed with non-parametric Wilcoxon-signed-rank tests. Comparisons of both exposure conditions versus sham were performed for sessions 1 and 2, and the difference between the two sessions. Multiple endpoint adjustment for cognitive outcomes was performed as in Regel et al. (2007b), and the significance level was adjusted accordingly to P < 0.015. One subject had to be excluded from the analysis of the cognitive tasks because one session could not be started on time due to software problems.
Results
- Top of page
- Summary
- Introduction
- Materials and Methods
- Results
- Discussion
- Acknowledgements
- Conflicts of Interest
- References
Sleep variables and subjective measures
Spectral analysis of the sleep EEG revealed an increase of power in the spindle frequency range following exposure in the second NREM sleep episode [Condition, F2,58 > 3.42, P < 0.04 (NREM); F2,58 > 4.14, P < 0.03 (stage 2)]. Post hoc analyses revealed that this increase was significant in both stage 2 and NREM sleep following the 14-Hz pulse-modulation condition (P < 0.05: 12.75–13.25 Hz in NREM sleep; P < 0.05: 11.25, 12.75–13 Hz in stage 2 sleep; Fig. 2). The maximum increase for both NREM and stage 2 sleep was seen at 13 Hz, with a magnitude of 18% and 23%, respectively. Trend level increases (approximately 11%) were also observed in several adjacent frequency bins (P < 0.1: 11 and 11.5 Hz in stage 2 sleep). A similar increase in spectral power was observed following the 217-Hz pulse-modulation condition (approximately 16%); however, it did not reach significance.
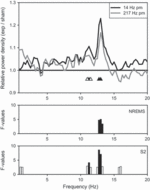
Figure 2. Average relative EEG power density spectra (derivation C3A2, 0.25-Hz bins, n = 29) for stage 2 sleep during the second non-rapid eye movement (NREM) sleep episode [14-Hz pulse modulation (pm): black; 217-Hz pm: grey] expressed relative to the sham condition (sham = 1.0). Statistical analysis revealed an increase within the spindle frequency range during NREM sleep (NREMS: 12.75–13.25 Hz; spectra are basically superimposed on the stage 2 spectra and therefore for clarity are not shown) and stage 2 (S2: 11.25 Hz and 12.75–13 Hz) of the second NREM sleep episode following the 14-Hz pulse-modulated condition. F-values (P < 0.05, black bars; P < 0.1, open bars) of the analysis with linear mixed model anova (factor Condition) are illustrated. Frequency bins following the 14-Hz pulse-modulated condition that were significantly different from sham (paired t-test) are indicated with black triangles (P < 0.05), trends with open triangles (P < 0.1).
The temporal evolution of the effect following the 14-Hz pulse modulation was analysed for the first four NREM sleep episodes in the affected frequency ranges in stage 2 sleep (slow spindle range: 11–11.5 Hz; fast spindle range: 12.75–13 Hz). Statistical analysis revealed a significant Condition effect (F1,203 = 4.75, P = 0.031) for the fast spindle range only. Post hoc analysis showed a significant increase of power in the second NREM sleep episode (P = 0.008; Fig. 3).
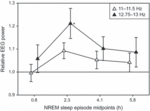
Figure 3. Temporal evolution of EEG spectral power (mean ± SEM) following the 14-Hz pulse-modulation condition relative to sham (sham = 1.0) in the first four NREM sleep episodes within the two affected frequency ranges (slow spindle range: 11–11.5 Hz, open triangles; fast spindle range: 12.75–13 Hz, black triangles) of stage 2 sleep. NREM sleep episode midpoints are indicated in hours after lights off (0.84, 2.32, 4.09 and 5.79 h). Linear mixed model anova followed by a post hoc paired t-test performed separately for the two bands showed a significant difference in the second NREM sleep episode for the fast spindle range only (*P = 0.008, paired t-test).
The effect was further explored for each individual’s spindle peak in the power density spectra (power ± 0.5 Hz around peak) in stage 2 sleep of the second NREM sleep episode. A relative increase or decrease was arbitrarily defined as a ≥5% change from sham. Following the 14-Hz pulse-modulated exposure, 62% (18/29) of subjects had an increased amplitude (maximal increase 76%), while 24% (7/29) showed a decrease in power compared with sham (maximal decrease 24%; Fig. 4). Individual spindle peak power following the 217-Hz pulse-modulated exposure showed both increases (11/29, 38%) and decreases (13/29, 45%) compared with sham, but there was no association with the respective change in the 14-Hz pulse-modulated condition. The specificity of the effect was also investigated; however, there was no correlation between the distance of each individual’s spindle peak frequency from 14 Hz and their respective increase or decrease in spindle peak after exposure to the 14-Hz pulse-modulated field.
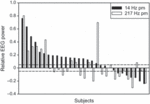
Figure 4. Individual change in spindle peak power (±0.5 Hz around individual peak location) relative to sham (=0) in stage 2 of the second NREM sleep episode for the 14-Hz and 217-Hz pulse-modulation (pm) conditions. The two dashed lines indicate a ±5% change.
Analysis of spectral data during REM sleep showed a few scattered significant P-values for both RF EMF conditions in the second and third REM sleep episode, and an increase of approximately 11% (11.75–12.25 Hz, P < 0.03, paired t-test) in the fourth REM sleep episode compared with sham for the 217-Hz pulse-modulated condition.
No significant effects on sleep architecture were found (Table 1). In particular, sleep efficiency, waking after sleep onset and REM sleep percentage/latency were not affected by exposure. The amount of NREM and REM sleep for each cycle, as well as the contribution of stage 2 sleep to NREM sleep, is shown in Table 2. No exposure-related changes were observed. Additionally, heart rate, as derived from ECG data (R–R intervals), was analysed separately for each NREM and REM sleep episode and showed no exposure-related alterations.
Subjects were unable to identify the exposure conditions, with the majority of responses (64/90) reporting no perception of a field at all. Where subjects did report having perceived a field (14), they were mostly incorrect and no one was able to correctly identify all three conditions. Furthermore, no differences between exposure conditions were observed for subjective mood, wellbeing or sleep quality.
Cognitive tasks
A significant session effect (1st versus 2nd half of exposure) was seen in all cognitive tasks, independent of exposure condition. Overall, reaction speed tended to be lower across tasks during active exposure conditions compared with sham (Fig. 5); however, this was not always the case. After correction for multiple comparisons no significant differences in speed were observed in the SRT and CRT. In the 1-back and 2-back tasks, speed was significantly affected by exposure (Condition, 1-back: F2,145 = 6.64, P = 0.002; 2-back: F2,145 = 7.52, P = 0.0008); however, post hoc analyses showed that there was only a trend level decrease in speed in the first session of the 2-back task for the 217-Hz pulse-modulated condition (P = 0.035). Accuracy was significantly decreased in the first session of the 3-back task in the 14-Hz pulse-modulation condition (P = 0.013, Wilcoxon-signed-rank test). No further effects on reaction speed or accuracy were observed.

Figure 5. Mean reaction speed (±SEM) for all five cognitive tasks performed during the 30-min exposure (n = 29) in the 14-Hz and 217-Hz pulse-modulation (pm) conditions and sham. Sessions indicate the first and second 15 min of exposure. In the 1-back and 2-back tasks, reaction speed in the active field conditions was different from the sham condition (mixed model anova, factor ‘Condition’; P < 0.015). However, post hoc analyses showed that there was only a trend level decrease in speed in the first session of the 2-back task for the 217-Hz pulse-modulated condition (P = 0.035). (SRT, simple reaction time task; CRT, 2-choice reaction time task).
Discussion
- Top of page
- Summary
- Introduction
- Materials and Methods
- Results
- Discussion
- Acknowledgements
- Conflicts of Interest
- References
Consistent with previous research, the current study showed that pulse-modulated RF EMF alters brain physiology. Specifically, exposure led to an increase in EEG power during NREM sleep in the spindle frequency range, which has also been reported previously by a number of well-controlled studies (Borbély et al., 1999; Huber et al., 2000, 2002; Loughran et al., 2005; Regel et al., 2007b). The increase in EEG spectral power was present following the 14-Hz pulse-modulated exposure condition in both stage 2 and NREM sleep in the second NREM sleep episode. This suggests that the 14-Hz pulse-modulation component, which is in proximity to the physiological sleep spindle frequency, is one potential mediator of the observed effects on the sleep EEG.
The 14-Hz pulse modulation led to a significant increase in EEG spectral power; however, there was also a smaller but non-significant increase observed following the 217-Hz condition. This suggests that the specificity of the pulse modulation may not be the most important factor as, to a certain extent, both pulse-modulation frequencies resulted in an enhancement of EEG spectral power in the spindle frequency range during sleep. Thus, it may be that any biologically relevant pulse modulation of RF EMF (that is, pulse modulation in a physiological range) would be sufficient to induce changes in electrical brain activity. In support of this, an enhancement of frequency-related biological rhythms was reported by Bawin et al. (1973), who showed that exposure to high-frequency (147 MHz) EMF, amplitude-modulated at frequencies close to the biologically dominant frequency, acted as a reinforcer and increased the rate of occurrence of spontaneous rhythms. Furthermore, stimulation frequency-dependent effects on brain activity have also been observed with transcranial magnetic stimulation (for review, see Sack and Linden, 2003), while another recent study reported altered intracortical excitability specifically in relation to GSM mobile phone exposure (Ferreri et al., 2006).
The relative non-specificity of the applied pulse modulation could imply that, aside from the frequency of the pulse modulation, the power of the RF EMF may also play an important role in inducing changes to sleep EEG spectral power such as those observed in both the current study and previous studies. For example, as pulse peak power was almost four times higher for the 14-Hz pulse-modulated exposure (crest factor = 30.95) than for the 217-Hz pulse-modulated exposure (crest factor = 8), this could be partly responsible for the more prominent increase in sleep EEG spectral power observed following the 14-Hz exposure condition.
Further to the specificity of the pulse modulation and the applied power of the RF EMF, the possible contribution of higher harmonics in the applied signal can also not be excluded as a potential factor in the current study. Exposure consisted of pulse-modulation components of either 14 Hz or 217 Hz, plus the associated higher harmonics (Fig. 1). Therefore, the observed effect could depend on different frequencies or a combination of different frequencies to a certain extent. Further research employing exposures that attenuate these higher harmonics would help to clarify the impact of these signal characteristics in relation to the effects on the sleep EEG.
In terms of the temporal evolution of the effect, the enhancement of EEG spectral power was most prominent for both exposure conditions in the second NREM sleep episode, which corresponds to approximately 2–3 h after sleep onset and exposure cessation. This observed effect declined in the latter sleep episodes and, although still present, did not reach significance. These results provide further evidence that the effect of pulse-modulated RF EMF outlasts exposure by several hours. However, the time-course of the effect is a characteristic that has varied across previous studies, which have reported an increase of the effect towards the end of sleep (Huber et al., 2002), similar levels throughout sleep (Regel et al., 2007b), or that the effect is only present at the beginning of sleep and/or decreases in the course of sleep (Borbély et al., 1999; Huber et al., 2000; Loughran et al., 2005). It remains unclear why the temporal evolution of the effect has been so variable. The discrepancies could be due to a number of experimental factors that have differed between studies. For example, the different exposure parameters (such as duration and intensity of exposure, crest factors and modulation schemes) employed across studies or the influence of individual variability in response to exposure to pulse-modulated RF EMF could play a role. Indeed, there was considerable variability in the effect of exposure on spectral EEG power during sleep in the current study, with the majority of participants showing an increase, while some showed no change or even a decrease following the 14-Hz pulse-modulated exposure. The presence of individual differences in response to RF EMF exposure was also recently shown by Loughran et al. (2008), which highlights the importance of the consideration of inter-individual variability when conducting human research on RF-related biological effects.
Despite the changes observed in NREM sleep EEG spectral power following exposure, there were no effects on sleep architecture. This is consistent with the majority of previous studies, and confirms that the responses seen in the EEG do not result in any changes to the overall pattern or quality of sleep. In contrast to most previous research, it must be noted that some small effects on EEG spectral power during REM sleep were seen in the present study. Only one early study reported effects on REM sleep EEG power (Mann and Röschke, 1996), and this was unable to be replicated by the same researchers in subsequent studies (Wagner et al., 1998, 2000) or by other independent researchers (Borbély et al., 1999; Huber et al., 2000, 2002; Loughran et al., 2005; Regel et al., 2007b). There was no clear pattern in the small changes of EEG power observed during REM sleep, and therefore until independently verified or replicated, interpretation remains difficult.
Regarding effects on cognition, it is still unclear to what extent RF EMF influences cognitive performance, such as attention, reaction speed and working memory. In the current study reaction speed tended to be slower with 217-Hz pulse modulation in all tasks, whereas accuracy of performance was largely unaffected. A number of previous studies have investigated exposure-related effects on cognition, using the same or similar tasks, and although changes in reaction speed and accuracy have been reported, no consistent effects have been observed. This may suggest that there are no repeatable effects of pulse-modulated RF EMF on cognitive performance, or that the tests currently employed may not be sensitive enough to elucidate such effects (Regel and Achermann, 2011). Additionally, a recent review and meta-analysis by Valentini et al. (2010) highlighted the heterogeneity of results from previous studies, and concluded that there is currently no evidence that cognition is influenced by mobile phone-like RF EMF exposure.
In conclusion, our results provide further evidence for short-term effects of RF EMF exposure on the sleep EEG in healthy, young male adults. In particular, we showed that pulse-modulation frequency components within a physiological range are sufficient to induce these effects. Despite these repeatable short-term effects on the sleep EEG, there is no indication of an impact on overall sleep quality, neither objective nor subjective, or on cognitive performance. Further research on the specificity of pulse-modulation components may help to provide an insight into potential mechanisms behind the observed EEG alterations, which may also bring us closer to potential consequences of mobile phone use for public health.
Acknowledgements
- Top of page
- Summary
- Introduction
- Materials and Methods
- Results
- Discussion
- Acknowledgements
- Conflicts of Interest
- References
The authors thank Mona Neubauer, Melanie Achermann and Patricia Milz for their help during the experiment, Karl Wüthrich and Dr Roland Dürr for technical support, and Dr Sven Kühn for exposure-related support. This study was supported by the Swiss National Science Foundation (National Research Programme 57: ‘Non-Ionising Radiation – Health and Environment’).
Conflicts of Interest
- Top of page
- Summary
- Introduction
- Materials and Methods
- Results
- Discussion
- Acknowledgements
- Conflicts of Interest
- References
All authors declare no conflict of interest.
References
- Top of page
- Summary
- Introduction
- Materials and Methods
- Results
- Discussion
- Acknowledgements
- Conflicts of Interest
- References
- Effects of modulated very high frequency fields on specific brain rhythms in cats. Brain Res., 1973, 58: 365–384. , and
- Pulsed high-frequency electromagnetic field affects human sleep and sleep electroencephalogram. Neurosci. Lett., 1999, 275: 207–210. , , , , and
- Dosimetric evaluation and comparison of different RF exposure apparatuses used in human volunteer studies. Bioelectromagnetics, 2008, 29: 11–19. , , , , and
- Acute mobile phone operation affects neural function in humans. Clin. Neurophysiol., 2002, 113: 1623–1632. , , , , and
- The effect of mobile phone electromagnetic fields on the alpha rhythm of human electroencephalogram. Bioelectromagnetics, 2008, 29: 1–10. , , , , and
- Effects of 2G and 3G mobile phones on human alpha rhythms: resting EEG in adolescents, young adults, and the elderly. Bioelectromagnetics, 2010, 31: 434–444. , , et al.
- Is the brain influenced by a phone call? An EEG study of resting wakefulness Neurosci. Res., 2005, 53: 265–270. , , , , and
- Systematic trends across the night in human sleep cycles. Psychophysiology, 1979, 16: 283–291. and
- Mobile phone emissions and human brain excitability. Ann. Neurol., 2006, 60: 188–196. , , , , and
- Effects of short- and long-term pulsed radiofrequency electromagnetic fields on night sleep and cognitive functions in healthy subjects. Bioelectromagnetics, 2007, 28: 316–325. , , et al.
- Effect of a 902 MHz electromagnetic field emitted by mobile phones on human cognitive function: a replication study. Bioelectromagnetics, 2003, 24: 283–288. , , et al.
- 902 MHz mobile phone does not affect short term memory in humans. Bioelectromagnetics, 2004, 25: 452–456. , , et al.
- Exposure to pulsed high-frequency electromagnetic field during waking affects human sleep EEG. Neuroreport, 2000, 11: 3321–3325. , , et al.
- Electromagnetic fields, such as those from mobile phones, alter regional cerebral blood flow and sleep and waking EEG. J. Sleep Res., 2002, 11: 289–295. , , et al.
- Radio frequency electromagnetic field exposure in humans: estimation of SAR distribution in the brain, effects on sleep and heart rate. Bioelectromagnetics, 2003, 24: 262–276. , , et al.
- Exposure to pulse-modulated radio frequency electromagnetic fields affects regional cerebral blood flow. Eur. J. Neurosci., 2005, 21: 1000–1006. , , et al.
- International Commission on Non-Ionizing Radiation Protection. Guidelines for limiting exposure to time-varying electric, magnetic, and electromagnetic fields (up to 300 GHz). Health Phys., 1998, 74: 494–522.
- The effects of electromagnetic field emitted by GSM phones on working memory. Neuroreport, 2000, 11: 1641–1643. , , , and
- The effect of electromagnetic fields emitted by mobile phones on human sleep. Neuroreport, 2005, 16: 1973–1976. , , , , and
- Individual differences in the effects of the radiofrequency electromagnetic fields emitted by mobile phones on human sleep. In: 29th URSI General Assembly, Chicago, Illinois, USA, 2008. , , and
- Effects of pulsed high-frequency electromagnetic fields on human sleep. Neuropsychobiology, 1996, 33: 41–47. and
- Effect of a 915-MHz simulated mobile phone signal on cognitive function in man. Int. J. Radiat. Biol., 1999, 75: 447–456. , , et al.
- Manual of Standardized Terminology, Techniques and Scoring Systems for Sleep Stages of Human Subjects. National Institutes of Health, Bethesda, Maryland, 1968. and
- Cognitive performance measures in bioelectromagnetic research – critical evaluation and recommendations. Environ. Health, 2011, 10: 10. and
- Pulsed radio frequency radiation affects cognitive performance and the waking electroencephalogram. Neuroreport, 2007a, 18: 803–807. , , et al.
- Pulsed radio-frequency electromagnetic fields: dose-dependent effects on sleep, the sleep EEG and cognitive performance. J. Sleep Res., 2007b, 16: 253–258. , , et al.
- Combining transcranial magnetic stimulation and functional imaging in cognitive brain research: possibilities and limitations. Brain Res. Brain Res. Rev., 2003, 43: 41–56. and
- Neurophysiological effects of mobile phone electromagnetic fields on humans: a comprehensive review. Bioelectromagnetics, 2007, 28: 415–432. , , , , and
- Systematic review and meta-analysis of psychomotor effects of mobile phone electromagnetic fields. Occup. Environ. Med., 2010, 67: 708–716. , , , and
- Effects of radiofrequency electromagnetic fields on the human nervous system. J. Toxicol. Environ. Health B Crit. Rev., 2009, 12: 572–597. , , et al.
- Human sleep under the influence of pulsed radiofrequency electromagnetic fields: a polysomnographic study using standardized conditions. Bioelectromagnetics, 1998, 19: 199–202. , , , and
- Human sleep EEG under the influence of pulsed radio frequency electromagnetic fields. Results from polysomnographies using submaximal high power flux densities. Neuropsychobiology, 2000, 42: 207–212. , , et al.